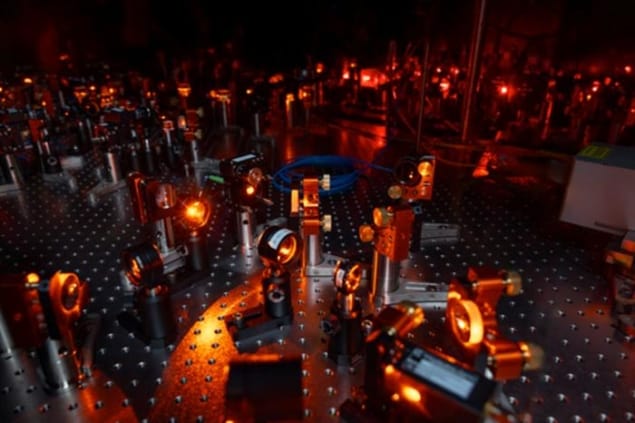
Ultracold molecules are a step closer to being a viable platform for quantum technology thanks to two independent teams of researchers who showed they could entangle pairs of molecules and encode them as quantum bits (qubits). Because molecules offer new ways to encode quantum information and can interact with each other over long distances, the two works offer new possibilities in quantum computing and quantum simulations beyond those provided by other types of qubits.
Quantum computation is different from classical computation. Whereas classical bits take values of either 0 or 1, qubits can be in a superposition of both. This makes it possible to perform certain complex calculations much more quickly, and the advent of fast and highly capable quantum devices is widely predicted to revolutionize computing.
That said, current qubit platforms such as trapped ions, quantum dots, Rydberg atoms and specially-designed superconducting circuits are restricted to “only†0s, 1s, and superpositions. Qubits made of molecules could change that. By definition, molecules consist of at least two atoms, and these atoms can vibrate and rotate with respect to each other. These vibrations and rotations create additional states for storing and processing information, meaning that three or more states could become available for computations.
Creating molecular qubits is no easy task, however, because these additional vibrational and rotational states must be tightly controlled. Among other consequences, this means that molecular qubits can only exist at ultracold temperatures, since any heat will cause the molecules to vibrate, rotate and translate so much that they decohere – meaning they lose their quantum nature and their ability to perform computations.
Opposites attract
The latest research, led by Lawrence Cheuk of Princeton University, US and independently by John Doyle and Kang-Kuen Ni of Harvard University, together with Wolfgang Ketterle at the Massachusetts Institute of Technology (MIT) and Eun-mi Chae from Korea University, focuses on molecules of calcium monofluoride (CaF). Because fluoride is such a strong attractor of electrons, these molecules are highly polar, which allows the opposite sides of two molecules to attract and interact via a mechanism called dipolar spin exchange.
This interaction leaves the molecules entangled, meaning that their quantum states are connected regardless of the distance between them. Information can then be encoded into the rotational states of the entangled molecules (the non-rotating part represents a 0 and the rotating part a 1) to create a two-qubit gate, which is one of the building blocks of quantum computing.
Molecool
To implement their two-qubit gates, both groups begin by laser cooling CaF molecules to microkelvin temperatures and using optical tweezers to create arrays of 20–40 individually-trapped molecules. At this point, the Princeton team take the extra step of making its molecular array defect-free by removing empty tweezer sites. Both groups then bring pairs of molecules to within micrometres of each other and allow them to interact. To avoid decoherence, the two groups select a particularly favourable set of rotational states to encode their qubits and apply microwave pulses to decouple the molecules from perturbations caused by interactions with their environment.
By precisely controlling the molecules’ interaction time, the two groups implement a two-qubit gate that brings the molecules into so-called Bell states. These states are maximally entangled, and the Princeton and Harvard-MIT teams created them with a fidelity (or quality) of 0.54 and 0.89, respectively.
The Princeton researchers further showed that this entanglement was created deterministically (that is, on-demand) and demonstrated the robustness of the Bell states by showing that they remain coherent even if the molecules are moved apart. The Harvard-MIT collaborators, meanwhile, demonstrated that they could control the rotational states of the molecules. By rotating the molecules 90 degrees, they showed they could alter the molecular interaction from antiferromagnetic to ferromagnetic and vice versa. This level of control makes it possible to probe the intermolecular interactions, which could be useful for simulating condensed-matter systems such as lattice spin models. Crucially, both teams succeeded in creating a maximally entangled two-qubit gate, which, when combined with single qubit rotations, is sufficient for universal quantum computing.
More complex molecules, higher fidelities
As a next step, Doyle says that his collaboration has already implemented a powerful technique called Raman sideband cooling to cool the molecules further, pushing them towards the motional ground state of the optical tweezer arrays that confine them. These lower temperatures should allow longer coherence times for the dipolar interaction. The collaboration is also planning to entangle more complex molecules like CaOH and SrOH. “We already worked out the necessary milestones before we can entangle these polyatomic molecules, such as laser-cooling and loading them into a magneto-optical trap, as well as creating an optical tweezer array of CaOH molecules,†he tells Physics World.
Cheuk, of Princeton, says his team has several plans for the immediate future. On a technical level, the team’s to-do list includes improving the coherence times of the molecules, the fidelity of preparing the molecular qubits, and the fidelity of the two-qubit gates. On the scientific side, because they have established the key building blocks of preparing and entangling individual molecules, they are interested in using their molecular arrays as a new platform to simulate larger-scale systems. “Typically, this concerns quantum many-body systems that could describe how different materials behave,†he says.
Molecular qubits stick around for longer
Phil Gregory, a physicist at Durham University, UK, who was not involved in either team’s work, says that both experiments display “remarkable control†over the CaF molecules. In his view, their biggest achievement is directly observing the dipolar spin exchange between two molecules, and he suggests this will be highly important for building stronger links between the quantum computing community and scientists who study ultracold molecules. The next step, he says, will be to increase the fidelity of state preparation and gate operation closer to 0.99 or even 0.999, which are the values needed to make a viable platform for quantum computing. Cooling the molecules further, he adds, should boost these fidelities and produce a significant improvement in gate performance.
The two experiments are described in back-to-back papers in Science.